- Details
A sphere that makes electrons oscillate
Plasmas play a vital role in many industrial applications. The energetically excited gases can be used, for example, to deposit coatings on surfaces, such as scratch-resistant protective layers on plastic spectacle lenses, or high-precision optical filters on quartz glass. In the process, the plasma is used to bombard the coatings that grow on the substrate by vapour deposition with ions, thus effectively hammering them into place.
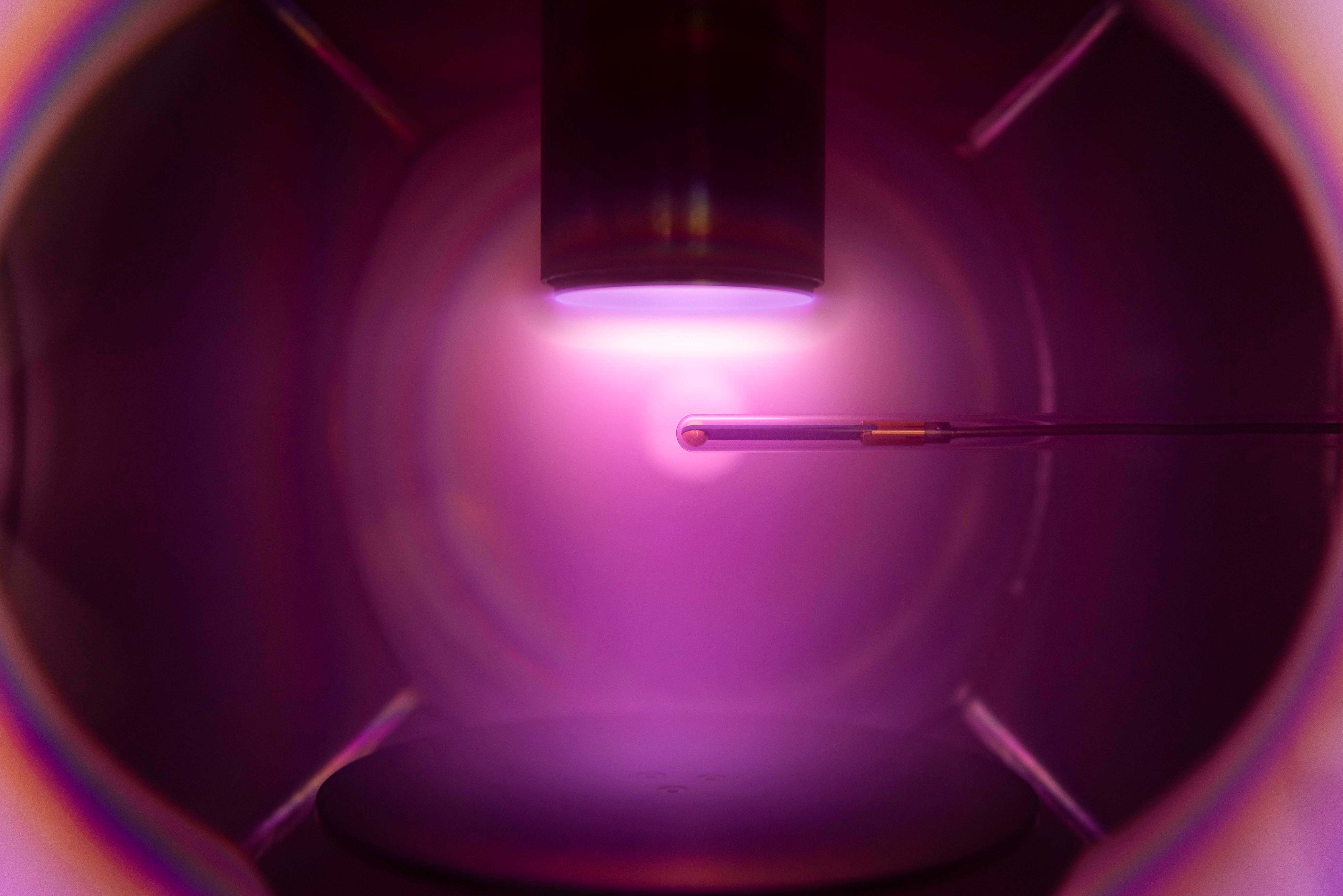
But such processes must meet many requirements. For example, they must take place at low temperatures to prevent damage to the coated surfaces. “In modern production processes, more and more emphasis is also being placed on precision,” stresses Professor Ralf Peter Brinkmann, who holds the Chair for Theoretical Electrical Engineering at RUB. All resulting products must be exactly the same, and the coating must be defect-free. To achieve this level of precision, it is necessary to constantly monitor the plasma. The electron density is particularly important in coating processes. If it were to fluctuate too much, this would negatively affect the quality of the finished coating. “Ideally, the electron density should be constantly measured and automatically readjusted if necessary, so that no human has to interfere in the process,” explains Brinkmann.
Small, reliable, maintenance-free
A measuring instrument that can do all this has to meet a variety of requirements: It should be as small as possible, reliable, maintenance-free, and it must neither interfere with the coating process nor be damaged in the plasma. Experts refer to this as “process-compatible plasma diagnostics”.
Researchers have been pursuing one idea for a long time: The electrons, which move freely in the plasma, can be made to oscillate by applying a small external voltage. If the right frequency is hit, a detectable resonance occurs. Since the resonance frequency depends on the electron density, this can then be theoretically calculated.
Picture this like driving an old car.
– Ralf Peter Brinkmann
However, earlier attempts to put this idea into practice encountered some difficulties. A Japanese research group, for example, proposed a very simple construction: The team used a coaxial cable, similar to an analogue antenna cable, allowed the inner conductor to protrude a little, and inserted the end of the cable into the plasma. If a voltage was applied, resonances of the plasma could be measured. However, resonances of equal value occurred at several different frequencies – a veritable zoo. “The problem was: which one should be used for diagnostics,” explains Ralf Peter Brinkmann.
Analyses by the Bochum-based researchers provided an answer to the question of where the different resonances came from: As simple as the design of the measuring equipment was, different oscillations with different resonance frequencies arose at different sections of the apparatus. “Picture this like driving an old car,” as Ralf Peter Brinkmann illustrates the principle. “At a certain speed, the exhaust rattles, at another, it’s the wing mirror.”
The more symmetrical, the better
To remedy the situation, the team devised a concept that aimed for the simplest possible oscillations. Their aim was: the more symmetrical, the better. “The spherical shape is the simplest configuration imaginable,” says Brinkmann. “A floating marble would’ve been our first choice.” However, it was not quite that simple, of course. Electricity always needs a forward and a return conductor. Accordingly, two metallic hemispheres were chosen. The construction is rotationally and mirror-symmetrical, both electrodes are the same size. “Here, too, we measure resonances at different frequencies,” explains Ralf Peter Brinkmann. “But they can be clearly sorted. The strongest resonance is the dipole resonance; the other, weaker ones, represent the overtones to this keynote, so to speak.” The name “multipole resonance probe” (MRP) was derived from the mathematical method of “multipole analysis” used for this purpose.
The reason why researchers opt for the MRP is that the relationship between plasma density and resonance frequency is given by a simple mathematical formula. The plasma density is the only unknown in this equation. Once the equation is solved, it can be calculated from the measured values. “This means that it is not necessary to calibrate the measuring probe before use, i.e. to compare it with other measured values,” elaborates Ralf Peter Brinkmann.
Plasma was kept consistent through constant monitoring
So much for the underlying theory. For practical implementation, the researchers teamed up with three other chairs at the faculty. Professor Ilona Rolfes’ High Frequency Engineering Institute carried out a high-frequency optimisation of the entire system consisting of probe head and holder. For example, it was possible to make the holder practically invisible to the plasma. Professor Thomas Musch’s Institute of Electronic Circuits designed control electronics based on radar technology. And finally, Professor Peter Awakowicz’ Institute for Electrical Engineering and Plasma Technology tested the finished probe in a number of plasma processes.
The Federal Ministry of Education and Research funded the joint projects Pluto and Pluto plus to develop the MRP to the point where it could be used in practice. This also provided the opportunity to test the probe with industrial partners. And it turned out that if the electron density in the plasma was kept consistent through constant monitoring by means of MRP and automatic adjustment of the control, the fluctuations in the process results were significantly reduced.
Today, a spin-off is about to get off the ground.
adapted from Meike Drießen (RUB)
- Details
Where basic science meets technology
Plasma physics is the study of the behaviour of ionised gases. Statistical physics, fluid dynamics, electrodynamics as well as atomic and molecular physics come together to form a discrete discipline. Plasmas determine both stellar evolution on astronomical scales and etching of nanostructures in the semiconductor industry. Plasma-based engines are already powering satellites in space, and very hot magnetised plasmas may provide clean energy through controlled nuclear fusion in the future. Tiny cold plasmas at atmospheric pressure offer a wide range of applications, from CO2 conversion to medicine and biology. Major advances in measuring the internal parameters of plasmas and in their simulation have recently contributed to a much better understanding of these complex systems. Wherever the journey into the future takes us, it will not be without plasmas.
by Uwe Czarnetzki (RUB)
- Details
A reference source for plasma research
The areas of application for plasmas seem inexhaustible. In biomedicine, for example, the technology promises to heal chronic wounds better, kill antibiotic-resistant germs or treat cancer cells selectively. “There are many studies that show that plasmas could be useful for these applications,” says Professor Judith Golda, research physicist in Collaborative Research Centre 1316. “But in order to use plasmas for these purposes, we need to understand their underlying mechanisms.”
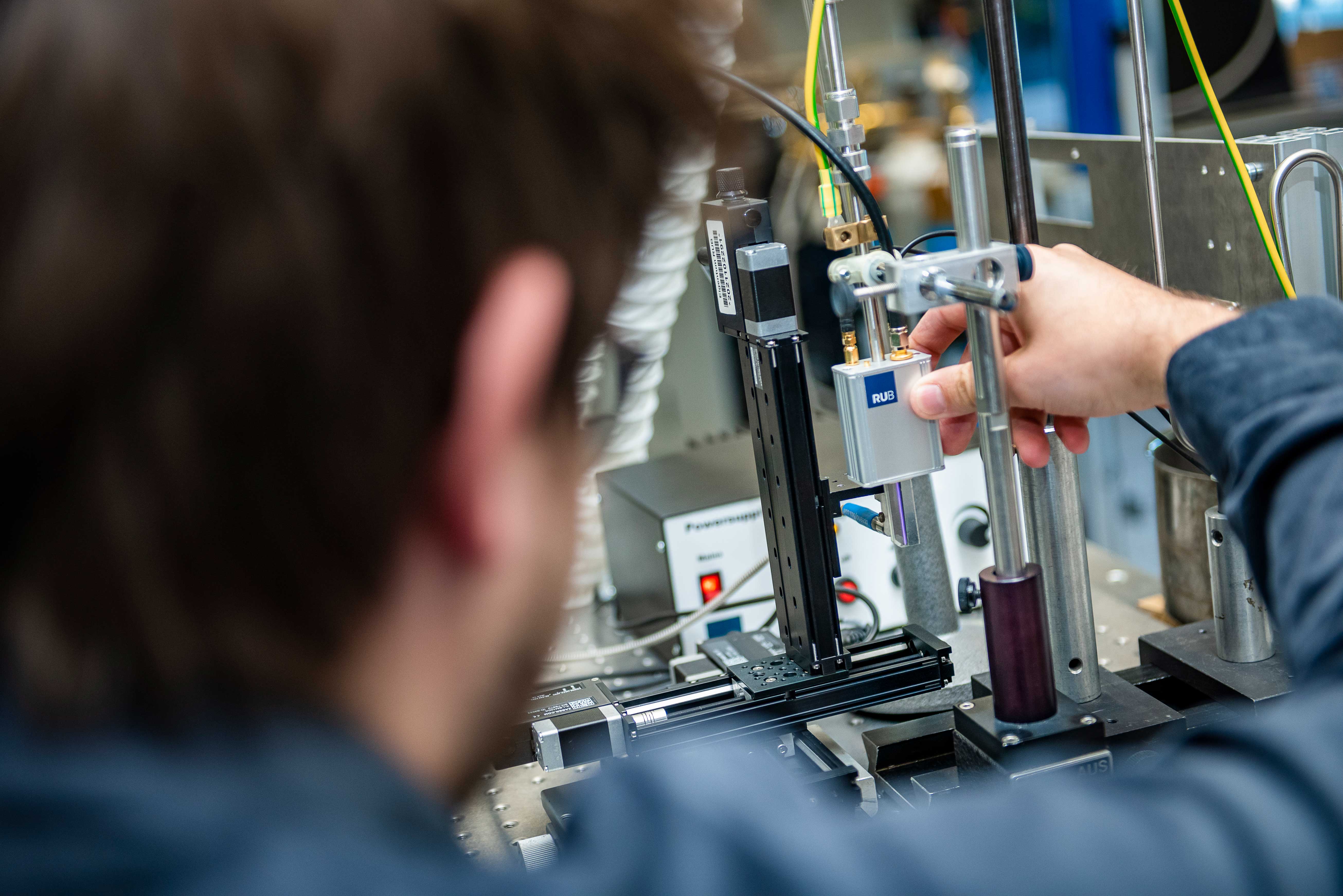
One problem is that while many studies have been done over the years, the techniques have not been comparable. “Making a plasma at atmospheric pressure is easy,” Golda explains. “All you really need are two wires to which you apply a voltage so that an electric field is created that ionises the gas between the wires.” Because it’s so easy, many research groups have built their own plasma sources. But not all plasma is the same. There are many properties that determine how suitable a plasma is for a particular purpose, such as the strength of the electric fields or the type and quantity of reactive particles it contains.
Complex plasma physics meets complex biological processes
Since both plasma physics and the biological processes that researchers want to manipulate with plasmas are very complex, it is difficult to explain the observed effects of plasma treatments mechanistically given the large number of variable parameters in plasma. In the early 2010s, therefore, the idea arose to develop a reference source, i.e. a plasma whose properties are precisely characterised and which can be reproduced with exactly these properties. The hope was that if different research groups used this plasma, they would know exactly which plasma parameters were responsible for certain effects. “Such a reference source already existed for low-pressure plasmas. We now wanted to transfer the concept to atmospheric-pressure plasmas,” recalls Judith Golda, who already worked on this issue while doing her PhD at RUB.
Together with partners from Greifswald, Eindhoven, Milton Keynes, York and Dublin, the Bochum group selected a plasma source that RUB physicist Dr. Volker Schulz-von der Gathen had already studied in-depth. “An important argument in our selection process was that many other groups worldwide have already worked with similar sources. So, we were looking for a plasma that could be used by the majority, so to speak,” explains Judith Golda.
Analyses at five locations yield the same results
The international team characterised the plasma source in detail. In the first step, the RUB researchers built five identical sources so that each cooperation partner could examine the plasma at their own location to ensure that it always had the same properties – which worked out well right away. The work took place within the framework of a funding programme of the European Cooperation in Science and Technology (COST). Thus, the researchers eventually dubbed their source COST-jet. Jet, because the plasma emerges from an opening in the form of a jet.
To make the plasma source available to all research groups worldwide, the project team wrote detailed assembly instructions and published them online at cost-jet.eu, where they can be freely accessed. “However, because it is sometimes not so easy for researchers without prior experience to implement such instructions, we also made sure that the COST-jet could be purchased ready-built at cost price,” points out Judith Golda. Sales are handled by Plasma Applications Consulting, a RUB spin-off.
Reference source deployed worldwide
Many groups worldwide are now using the COST-jet, so the research done by all these groups is comparable. Theoretically working groups complement this research.
“There are, of course, alternative sources,” says Judith Golda. “And they also have their justification, because you need different plasmas depending on the application.” Nevertheless, the research physicist is pleased that the reference plasma developed in Bochum is deployed at many plasma research locations around the world.
At RUB, too, research is ongoing in this field under the umbrella of the plasma Collaborative Research Centres. Groups from the fields of physics and electrical engineering are cooperating, for example, to understand in detail which reactive species form in the COST-jet and how their quantity changes with increasing distance from the source. “Depending on which gas you use, up to 1,000 different reactions can take place in a plasma in quick succession, because the reactive species interact in many combinations,” outlines Golda. The COST-jet is based on the noble gases helium and argon with small admixtures of other gases, for example oxygen and nitrogen. If these are dissociated and ionised in the electric fields, the atoms can take on all kinds of reactive forms: Oxygen, for example, can exist as a positively (O+) or negatively charged ion (O-), as a neutral atom (O), as molecular oxygen (O2) or ozone (O3). If nitrogen (N) is added to the mixture, numerous other combinations quickly emerge, for example NO, N2O, NO2 and others. “The reaction chemistry literally explodes,” as Golda describes the process.
Spectroscopic analysis of reactive species
The Bochum-based researchers were interested, for example, in how many reactive oxygen atoms are produced in the COST-jet and how their quantity decreases with increasing distance from the source. “Since many biological systems need a certain volume of atomic oxygen, plasma treatments with reactive oxygen species can have positive effects,” elaborates Judith Golda. “But sometimes it can be too much oxygen.” According to her, it is therefore important to know exactly how much reactive oxygen is present in the plasma and what the optimal distance from the target surface to the plasma would be. “This is the only way to later develop applications that are also safe for patients,” says the researcher.
Spectroscopy was used to make the necessary measurements. In the process, the researchers transmit laser light of a specific wavelength into the plasma. This light is absorbed by the oxygen particles, raising them to a higher energy level. After a while, they return to their ground state, emitting light of a certain wavelength, which the researchers can measure. The emitted wavelength depends on the particle that emits the light; a neutral oxygen atom, for example, sends back different light than a positively charged oxygen ion. Based on the amount of light emitted at certain wavelengths, the researchers can thus deduce the amount of different oxygen species.
We know pretty much what comes out of the source – that is only the case with a few sources.
– Judith Golda
This is how the team found that the amount of oxygen atoms in the plasma decreases exponentially as the distance from the source increases. Using analytical models, they also showed why this is the case. “Because the particles are so reactive, they quickly react further to form other compounds, such as molecular oxygen or ozone,” explains Judith Golda. The team is also researching the reactive species that result from adding nitrogen to the source, for example nitrogen monoxide (NO). This is particularly interesting for the researchers, because NO also occurs in the human body as a messenger substance and is important for wound healing. For example, they determine the flow pattern of NO molecules when they hit a surface from the plasma source.
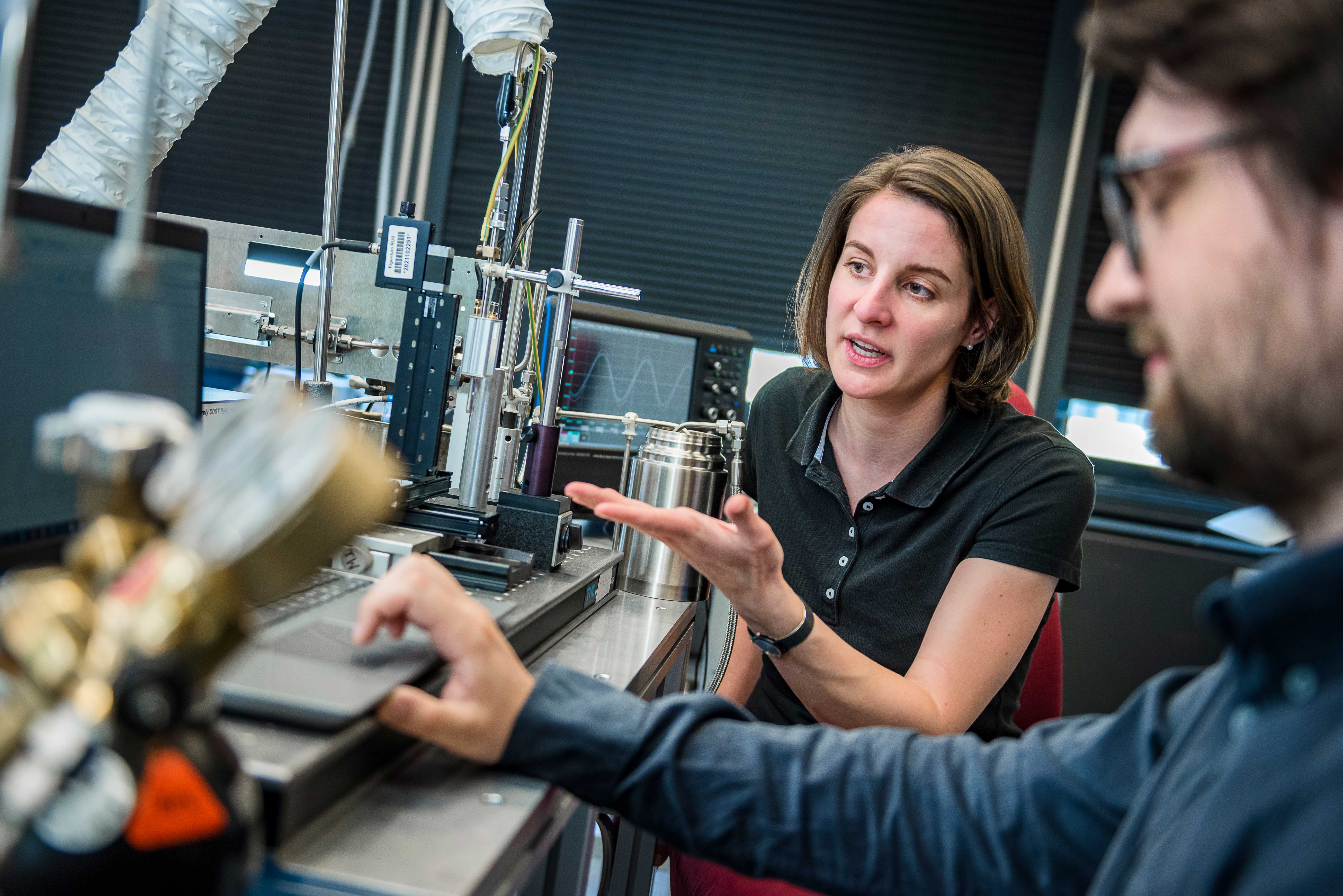
Overall, the COST-jet is already very well characterised, according to Judith Golda. “We know pretty much what comes out of the source – that is only the case with a few sources,” she says.
adapted from Julia Weiler (RUB)
- Details
Achieving success together
One of the most interesting and important features of plasma medicine as a topic is its interdisciplinary nature, bringing together natural scientists, engineers and clinicians. The number of different scientific areas represented within the field has been continuously growing in the past ten years. This interdisciplinary environment has been a driving force behind clinical studies in a number of cases, wound treatment being a prominent example.
Over the next ten years, as plasma medicine continues to grow and attract new researchers, I expect this trend to accelerate and for the field to become even more interdisciplinary than it is today. This will bring new perspectives on existing questions, new therapeutic concepts, and novel research methods. I believe that these factors will be crucial for new scientific discoveries and the translation of emerging approaches such as plasma-based cancer therapy, into clinical practice.
by Julia Weiler (RUB)
- Details
Plasmas for all
Driving the plasma van to school
For many years, the plasma researchers at RUB have been committed to introducing plasmas to school students in different year groups. “Physics teachers sometimes conduct experiments that involve plasmas, but the word plasma doesn’t even appear in the curriculum,” explains Science Manager Dr. Marina Prenzel. In order to familiarise secondary school students with the concept of a plasma, the SFB team, in cooperation with Professor Heiko Krabbe and other physics didactics experts, has constructed various plasma experiments that can be stowed away in boxes and handily transported in a minibus. The researchers use them for interesting 90-minute workshops in sixth-form classes, where students can do their own experiments and learn about different applications of plasmas. “This is how we want to create awareness that plasmas are extremely important for many of our current technologies,” says Prenzel.
Students evaluate research projects
Students should not only be given the chance to learn what a plasma actually is and where it is used. Rather, the SFB team is also currently setting up a project in collaboration with the physics didactics department that aims at promoting the evaluation skills of adolescents and young adults. Here, students are to gain insights into various plasma research projects and evaluate which of these projects they would support. Another goal is to convey the significance of plasmas for the challenges of global warming.
More than 20 years of plasma summer school
For more than 20 years, plasma researchers at RUB have been organising an annual international summer school for Master’s students and doctoral candidates. It originally emerged from a European Erasmus project, acquired under the auspices of the Eindhoven University of Technology. When the funding ran out in 2000, the RUB team dedicated itself to continuing it. “The school is practically always overbooked,” says co-organiser Dr. Marc Böke. The 80 to 90 participants each year and the lecturers come from all over the world. The aim of the seven-day school is to give them insights into all the major technically relevant plasmas and, at the same time, to enable them to network with each other and with established researchers in the field. “Some of the former participants are now themselves running plasma labs,” says Böke. The RUB team hopes to resume the successful format soon, despite the coronavirus situation.
Virtual tours through plasma labs
Even though direct contact with the public is very limited during the coronavirus pandemic, the SFB team came up with a solution: The researchers made 360-degree recordings of their laboratories. This means that not only school classes, but also interested members of the public have the opportunity to take part in guided tours of the laboratories.
On 27 October 2021, the SFB 1316 team offers a virtual public tour free of charge. Registration is possible via email (
adapted from Julia Weiler (RUB)
- Details
Using plasma and electrolysis for CO2recycling
The plasma ignites with a bright flash and tears through the water for a few billionths of a second. Dr. Katharina Grosse from Collaborative Research Centre 1316 “Transient atmospheric plasmas – from plasmas to liquids to solids” (RUB) takes spectacular pictures that show the ignition process of plasma under water at high temporal resolution. Delivering the first data sets with very high temporal resolution, the researcher supports a hypothesis on the ignition of these plasmas: there is not enough time in the nanosecond range to form a gas environment. The nanosecond plasma ignites directly in the liquid. The particles created during ignition can interact efficiently with catalytic surfaces.
But how does the plasma ignite in these short time scales? What happens afterwards? Which substances are produced? And how is this ignition in the liquid possible in the first place? In her doctoral thesis, physicist Grosse explores these very questions. To this end, she applies a high voltage to a hair-thin electrode immersed in water for ten nanoseconds. The strong electric field thus generated leads to the ignition of the plasma. Using high-speed optical spectroscopy in combination with modelling of the fluid dynamics, the Bochum-based researcher is able to predict power, pressure and temperature in these underwater plasmas and, consequently, to explain the ignition process and plasma development in the nanosecond range.
Hotter than the sun
This is what she has observed: at the time of ignition, extreme conditions exist in the water. For a short time, pressures of many thousands bar are created; this corresponds to or even exceeds the pressure at the deepest point in the Pacific Ocean, as well as temperatures of many thousands degrees, similar to the surface temperature of the sun. “In addition, a power of several 100 kilowatts is consumed in the plasmas for a short time, which more or less equals the connected load of several single-family houses,” explains Professor Achim von Keudell, Grosse’s doctoral supervisor and head of the Institute for Experimental Physics II.
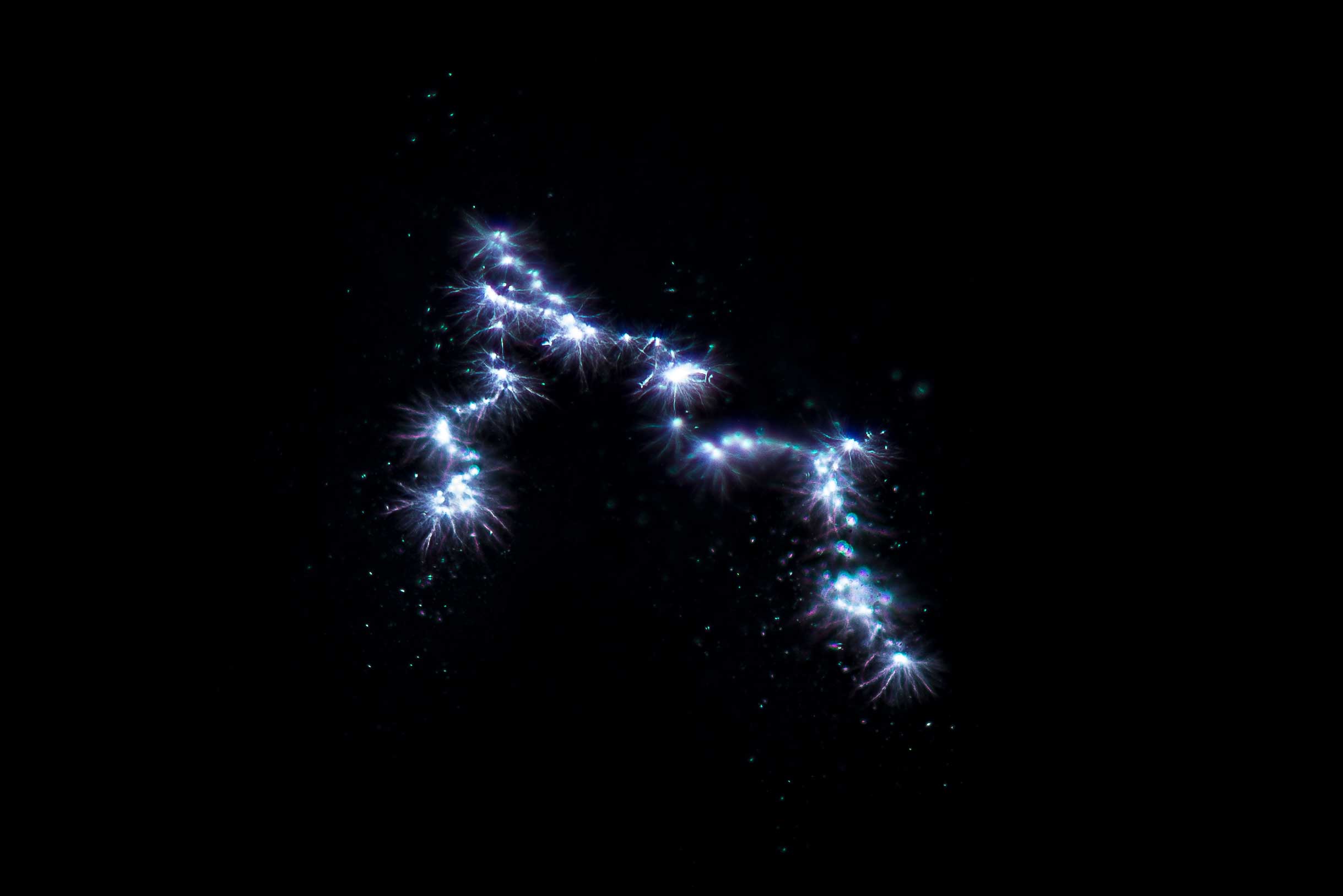
To achieve these measurement results, a complicated setup is necessary, which Katharina Grosse spent roughly a year on developing: “The electromagnetic interference is very strong and affects all measurement electronics. We had to build a large metal cage around the plasma chamber to bypass this source of interference. Another difficulty was to ensure the simultaneity of spectroscopic measurement and camera recording.”
Rendering plasma development visible
The tinkering has paid off: it is now possible to observe the plasma development very precisely. The recordings call into question the theory that has been common so far. Until now, this theory assumed that a high negative pressure difference forms at the tip of the electrode, which leads to the formation of very small cracks in the liquid with expansions in the nanometre range, in which the plasma can then spread. “It was assumed that an electron avalanche forms in the cracks under water, enabling the ignition of the plasma,” says von Keudell. However, the images taken by the research team from Bochum suggest that the plasma is “ignited locally within the liquid,” explains Grosse.
Tunnel effect under water
In her attempt to explain this phenomenon, the physicist uses the quantum-mechanics tunnel effect. This describes the fact that particles are able to cross an energy barrier that they should not be able to cross according to the laws of conventional physics, because they do not have enough energy to do so. “If you look at the recordings of the plasma ignition, everything indicates that individual electrons tunnel through the energy barrier of the water molecules to the electrode, where they ignite the plasma locally, i.e. precisely where the electric field is highest,” says Grosse. This theory has a lot going for it and is the subject of much discussion among experts. Subsequent experiments with negative pulses are to support Grosse’s tunnel theory.
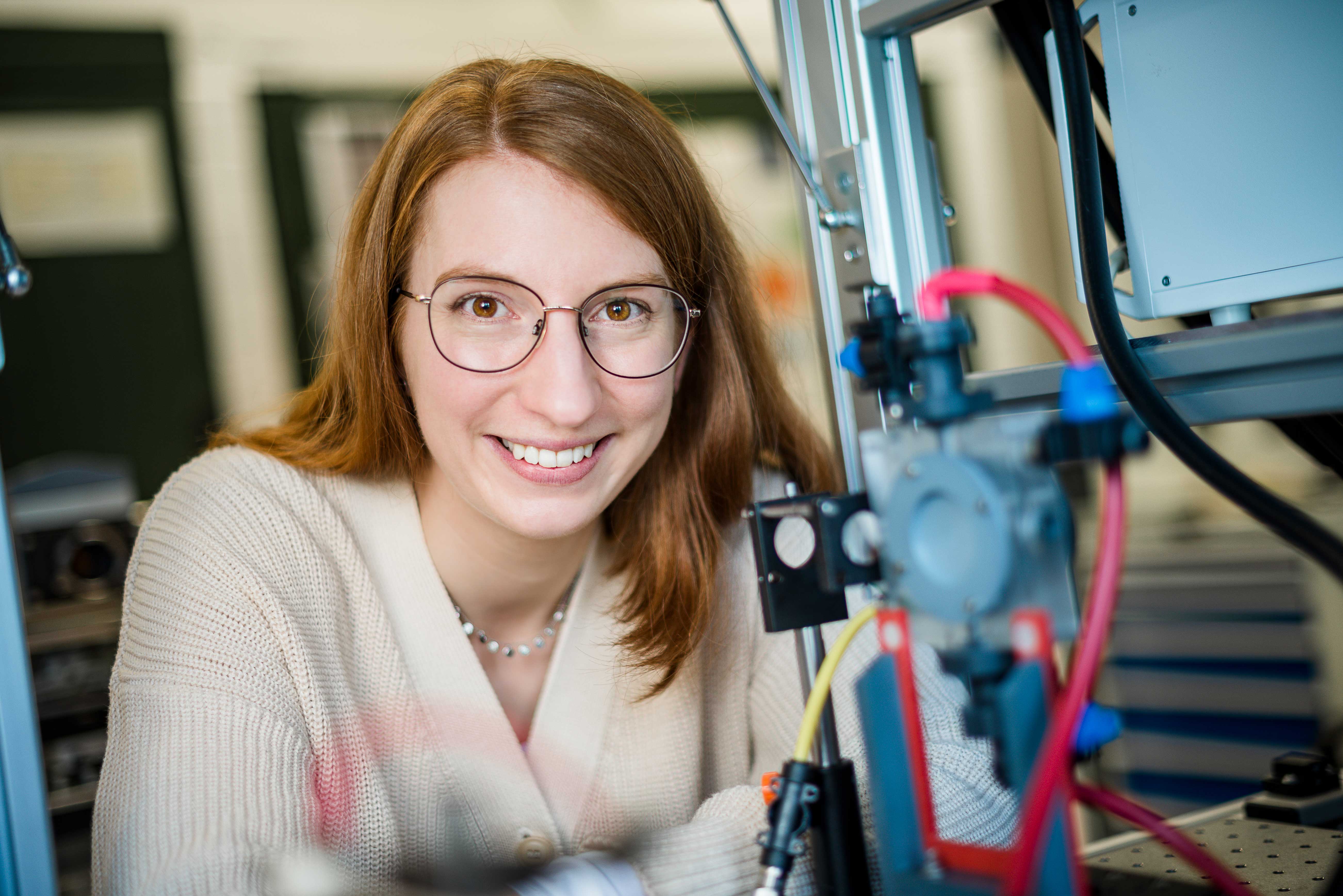
Water is broken down into its components
The ignition process under water is as fascinating as the results of the chemical reaction are promising for practical applications. The emission spectra show that, at nanosecond pulses, the water molecules no longer have the opportunity to compensate for the pressure of the plasma. The plasma ignition breaks them down into their components, namely atomic hydrogen and oxygen. The latter reacts readily with surfaces. And this is precisely where the great potential lies, explains physicist Grosse: “The released oxygen can potentially re-oxidise catalytic surfaces in electrochemical cells so that they are regenerated and once again fully develop their catalytic activity.”
Underwater plasmas and electrolysis
How exactly is this going to be achieved? Can plasma and electrolysis be combined? RUB PhD student and chemist Philipp Grosse is looking for answers to these questions at the Fritz Haber Institute of the Max Planck Society in Berlin. “Electrochemical cells,” he explains, “help, for example, to reduce, recycle and convert carbon dioxide into useful chemicals. This requires a catalyst. However, during the electrochemical process, the catalytic surfaces wear out and lose their catalytic capabilities.” This is where the underwater plasmas studied by Katharina Grosse could provide a remedy and be used for material conversion at the electrode-liquid interface.
The brother and sister team intends to find out in what way underwater plasmas can be used in the electrolysis of chemical substances. How can the plasmas support electrolysis by changing the liquid and the electrode surface? How does plasma interact with the electrochemical cell? For this purpose, Katharina Grosse is setting up her experimental setup in Berlin, where her brother Philipp has been conducting research for two years. Instead of water, they use electrolytes as liquids, and a catalytic surface is built directly into the plasma chamber. The Grosses decided to use copper oxide in the form of nanocubes as a catalyst. These are nanometre-sized copper oxide cubes that are used as a catalyst for CO2 reduction. They then apply a high voltage to the electrode for a few microseconds. A plasma ignites. The changes observed in the copper cubes suggest that the oxygen produced by the plasma ignition activates the copper oxide. The initial measurements imply that the extreme plasma is indeed able to re-oxidise the copper cubes and thus regenerate the catalytic surface. Once the catalyst is ready for use again, the electrochemical cell should also work, and with it the CO2 reutilisation process. This would allow CO2 to be continuously converted into other products in industrial plants; the cycle would thus be closed.
The dream of an infinite electrochemical cell
In Bochum and Berlin, the researchers are already dreaming of an infinite electrochemical cell in which electrochemical processes alternate with plasma ignitions. But the Grosses still have a long and complicated way ahead of them. The greatest challenge at present is to combine the physical with the chemical structure so that plasma ignition and electrolysis can take place simultaneously.
The future of plasma technology
Should this succeed, it would be a “milestone, a technology with a lot of potential,” stresses von Keudell. The chemical industry is very interested in such a plasma process, according to the spokesman for the Collaborative Research Centre: “They have high hopes for the electrification of the chemical industry.” The advantages of plasma technology: it takes up little space, and the electrical energy can support the conversion of chemical substances at the push of a button.
adapted from Julia Weiler (RUB)
- Details
Transforming climate killers into raw materials via plasma technology
Hydrogen, oxygen, carbon monoxide, carbon dioxide, methane – the steel industry releases a veritable cocktail of gases every hour. But how can these metallurgical gases be purified? This is where the research of Professor Peter Awakowicz from the Chair of Electrical Engineering and Plasma Technology and Professor Martin Muhler from the Lab of Industrial Chemistry comes in. The interdisciplinary research team at RUB studies how non-thermal plasma can be used for targeted cleaning and processing the metallurgical gas mixture. In the Carbon2Chem joint project funded by the German Federal Ministry of Education and Research (BMBF), in which both researchers have been involved since 2016, they are testing their innovative plasma technology on real gases. “The combination of a basic research project in Collaborative Research Centre 1316 and an application-oriented BMBF project has been a long-held dream of both of us that we can now fulfil,” says Awakowicz.
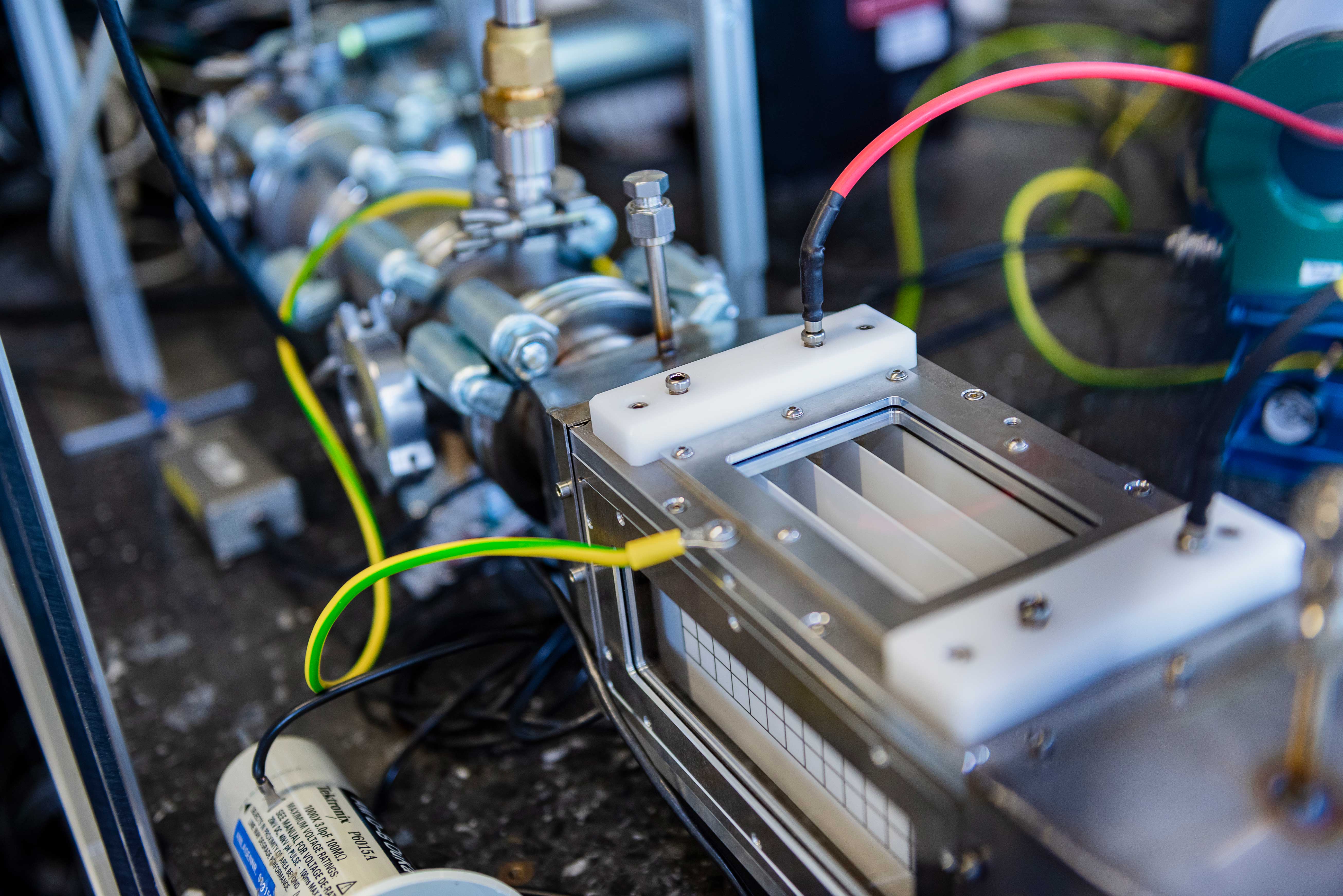
In sub-project L3 of Carbon2Chem, in which the RUB researchers are involved, the specific issue is pre-cleaning, the removal of oxygen from the coke oven gas. “This sounds simple, but it is tricky in detail,” explains research chemist Muhler. According to him, it is an intricate art to remove the oxygen from the predominantly hydrogenous coke oven gases. Traditional methods of exhaust gas purification, such as pressure swing adsorption, would not work if there was too much oxygen. The high chemical reactivity of oxygen would trigger dangerous gas reactions under normal pressure, such as an oxyhydrogen explosion. This is why Awakowicz and Muhler rely on pre-cleaning using plasma technology with cold plasma. How does it work? What makes non-thermal plasma so special? And how is it generated?
Innovative Technology for gas purification with cold plasma
Cold plasmas, or non-thermal plasmas, are plasmas in which the temperatures of ions, electrons and neutral particles vary. “The temperature of the electrons is high in these plasmas, while the temperature of the other gas particles is comparatively low,” explains Awakowicz. Since the plasmas are in thermal non-equilibrium, they are also often called non-equilibrium plasmas. They have an advantage with regard to gas purification processes: The ignited, cold plasma can be used for gas treatment without causing a significant increase in the temperature of the gas.
However, producing cold plasma is not easy. “The difficulty lies in supplying the gas with just enough energy so that the light electrons are accelerated and thus become hot, but the temperature of the large, heavy neutral particles and ions hardly changes,” explains Awakowicz. The research team from the Chair of Electrical Engineering and Plasma Technology has succeeded in producing precisely this state of non-thermal plasma in the purpose-built plasma reactor: The electrons become several tens of thousands of degrees Celsius hot, while the gas temperature of the entire plasma increases to barely more than room temperature.
“To achieve and understand this state, complex plasma diagnostics were necessary. We had to repeatedly readjust the individual parameters, such as the geometry and materials of the electrodes, the voltage amplitude and frequency, and associated with this the input power. Then the fundamental plasma parameters such as the electron density, the distribution function of the free electrons, but also the gas temperature had to be determined in order to optimise everything,” as Awakowicz describes the challenges.
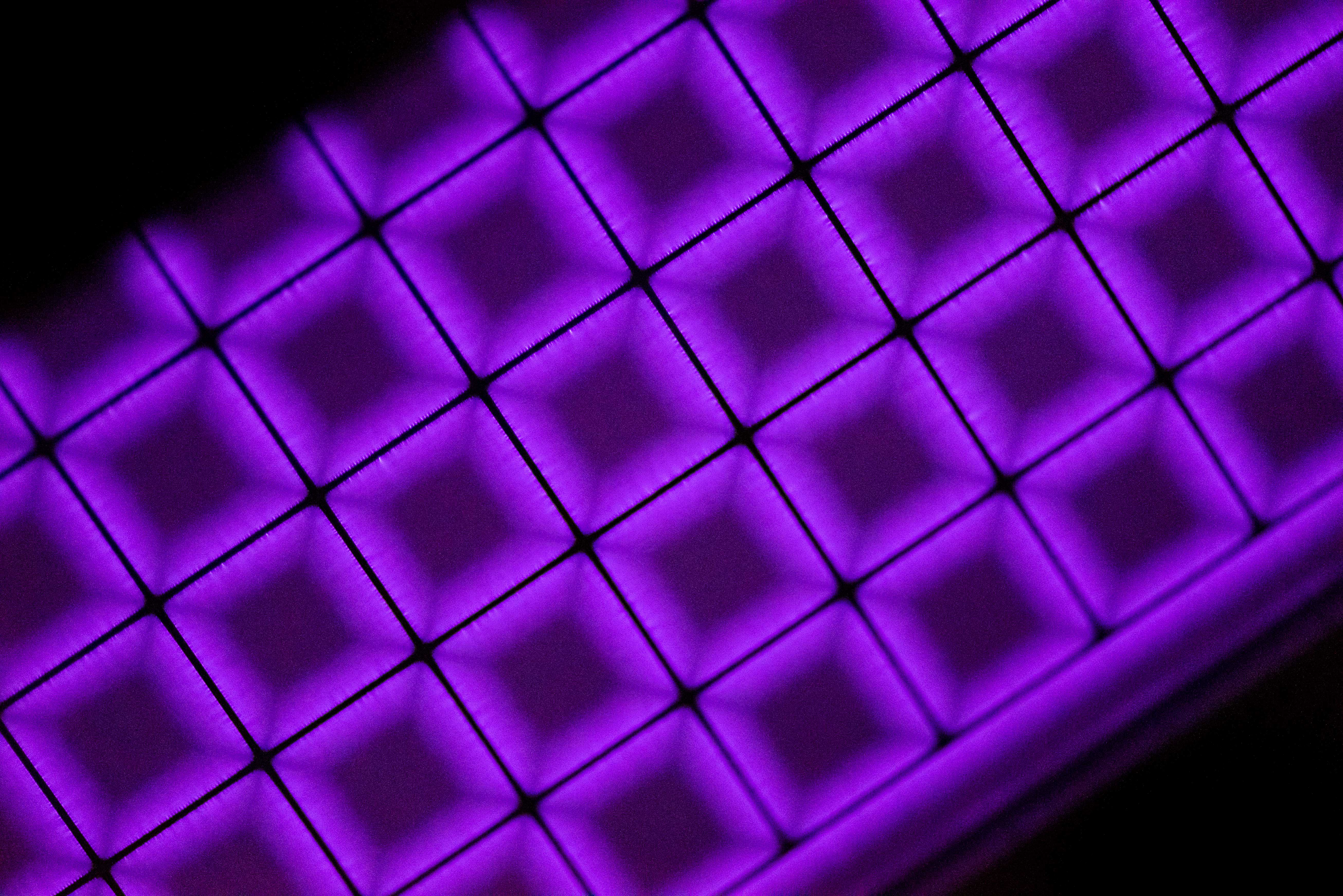
While the team led by electrical engineer Awakowicz was fine-tuning the parameters for producing the cold plasma, the chemical researchers led by Muhler were analysing the chemical reactions triggered by the plasma discharge. It turned out that the cold plasma is so reactive that it animates the oxygen contained in the coke oven gas to react with hydrogen, so that water is formed. The gas mixture is freed from oxygen and is thus ready for further purification processes.
Waste gas purification on an industrial scale
What Awakowicz and Muhler have fundamentally researched in the RUB laboratory is being applied to specific gas mixtures in the steel industry in the BMBF Carbon2Chem project. In the first project phase from 2016 to 2020, the researchers already provided proof of feasibility: Their plasma technology can be applied to these specific metallurgical gases. In the second funding phase from 2020 to 2024, the technical processes will now be further validated and scaled up for industrial application from 2025.
Scale-up in the Carbon2Chem pilot plant
The relevant experiments take place on an area of 3,700 square metres in the pilot plant in Duisburg. The pilot plant was built in 2018 adjacent to the thyssenkrupp Steel Europe site and means that Carbon2Chem’s experiments can be conducted under industrial conditions. “The real exhaust gases are routed to the pilot plant site, where they are available to us,” explains Muhler. “We now have to show that our plasma system can operate with the real gases – on a much larger scale, of course. The reactor should be able to purify more than fifty times the amount of gas,” as he outlines the challenge. At RUB, the researchers have so far worked with small gas flows of ten litres per minute in the lab; at the pilot plant, they are dealing with flows with a much larger volume of 500 litres per minute and more. “A spectacular project, since the dimensions are so huge,” point out Awakowicz and Muhler.
Industrial implementation planned in 2025
The commercial implementation of the gas purification plant is scheduled for four years from now. “The final step, scaling up from tenfold to one hundredfold, will be an effort,” Awakowicz suspects, adding: “As researchers, we will have to hand over the baton to industry at some point.”
adapted from Lisa Bischoff (RUB)
- Details
“That was a fairly crazy idea”
The synthesis of many chemicals results not only in the desired product but also in its mirror image: the physico-chemical properties of the so-called enantiomers are very similar and they are therefore difficult to separate. This, however is necessary, because they often have different biological properties. This is important, for instance, when it comes to drugs. The (S)-ibuprofen isomer is effective against pain, but its twin (R)-ibuprofen is not. “Occasionally, one of the two forms is even toxic,” Professor Julia Bandow, Chair of Applied Microbiology at the RUB Faculty of Biology and Biotechnology points out.
Her research group employs enzymes, i.e. biological catalysts derived for example from bacteria or fungi to produce such chemicals. Some enzymes produce only one of the two enantiomers.
Enzymes are sensitive
However, enzymes are in general rather sensitive catalysts. Some are susceptible even to inactivation by the substrate they convert. “This is the case for our example enzyme. The unspecific peroxigenase, or UPO for short, extracted from the edible fungus Agrocybe aegerita or chestnut mushroom and synthesised by the research group of Professor Frank Hollmann from Technical University Delft can produce the fragrance (R)-1-phenylethanol. It requires hydrogen peroxide as a substrate for this purpose. If hydrogen peroxide is simply added to the enzyme-containing solution in the form of a concentrate, the enzyme is quickly inactivated,” explains Julia Bandow.
This dilemma the team resolved using several tricks. One of them was to use a plasma to produce hydrogen peroxide on demand. “That was a somewhat crazy idea,” Bandow admits in retrospect. “Because in fact, plasmas are the preferred means of destroying things.” In plasmas, which are created by adding energy to a gas, numerous reactive substances are formed, for example atomic oxygen, hydroxyl radicals, free electrons, and various excited species. Consequently, plasmas can be used to inactivate cancer cells, biofilms, viruses, or prions. In this instance, however, the plasma was supposed to help protect the biocatalysts by providing the enzyme with exactly the right dose of hydrogen peroxide needed to catalyse the fragrance at the push of a button.
“The generation of hydrogen peroxide using plasma has advantages over alternative production methods by means of enzymes or electrodes. Such enzymes are expensive to produce, they are attacked by hydrogen peroxide and hydrogen peroxide production is difficult to dose. Biocatalysts like UPO can precipitate at electrodes and clog them,” explains Julia Bandow.
Trick number two
The group therefore experimented with plasmas based on air or noble gases ignited directly above the enzyme-containing solution to produce the fragrance (R)-1-phenylethanol. However, the enzymes at the surface still were quickly destroyed by the reactive species. Here, trick number two came into play: the researchers attached the enzymes to beads, small spheres with a porous surface that lie at the bottom of the solution and hold the enzymes in place. They tested the optimal composition of the beads beforehand, because not every enzyme can dock equally well on every surface and still do its job, as this sometimes requires enzymes to move.
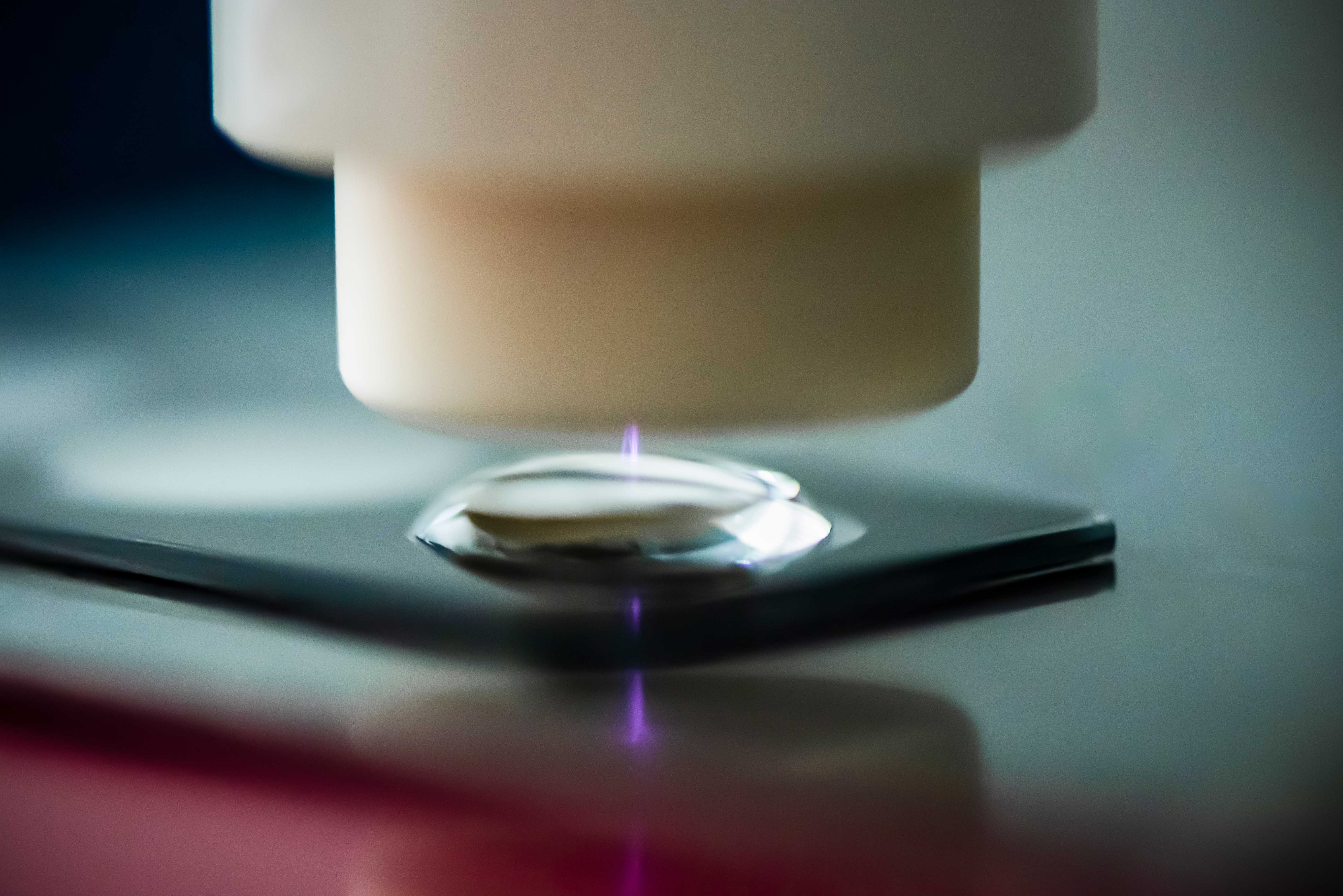
As a result, the beads sitting at the bottom of the container are covered by the solution, which acts as a buffer zone separating the enzyme from the plasma phase. The hydrogen peroxide produced by the plasma diffuses to the enzymes, where it is used in the reaction. Thanks to the buffer zone, the enzymes don’t come into contact with toxic doses of the substrate or other reactive species. Thus they remain intact and functional.
Tiny reactors
The reactors used so far are tiny. They can only hold up to five millilitres of liquid. “The protective layer that covers the beads is only about one millimetre thick,” says Julia Bandow. “This gap is sufficient to protect the enzymes from unstable and short-lived reactive substances that are created in the plasma. Some conveniently react to form hydrogen peroxide that is used as a substrate.” Hydrogen peroxide itself is comparatively long-lived. Its dose can be adjusted, for example, by pulsing the plasma, i.e. switching it on and off.
In the experiment, the enzymes were active for eight cycles of ten minutes without damage. Between cycles, the product (R)-1-phenylethanol was harvested and the second substrate, namely ethyl benzene, was replenished. The extraction of the product (R)-1-phenylethanol from the solution was carried out with ethyl acetate in just one step. “This is the great advantage of the biocatalytic over catalytic processes which produce both enantiomers,” stresses Julia Bandow.
Up to this point, the whole experiment was a proof-of-concept project, and it proved one thing: the approach works. Now, the research group is optimising the process, mainly with the aim of scaling up production volumes and optimising reactors. One reactor in which the enzyme-loaded beads rotate in the solution so that the enzymes have a steady substrate supply has already been tested successfully.
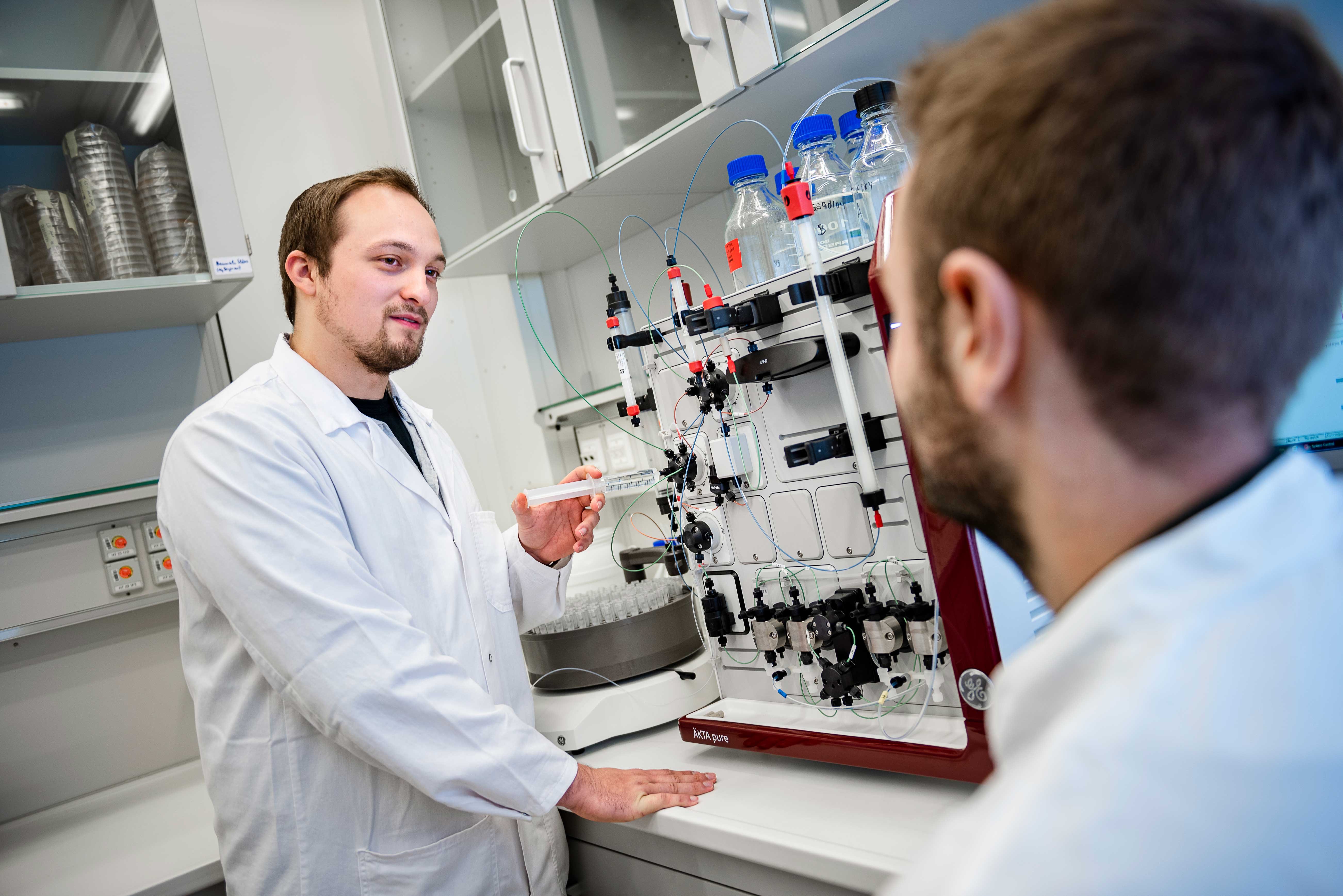
Further experiments have shown that the process works even better if the noble gas helium is used instead of air as the basis for plasma generation and if water vapour is added. “You find a lot more hydrogen peroxide in the solution, perhaps generated from plasma-generated OH radicals,” as Julia Bandow speculates. This increased the yield from about ten nanomole hydrogen peroxide per minute to 200 nanomole per minute. A further increase in hydrogen peroxide formation by 50 per cent was achieved by changing the voltage.
Our greatest success is that we have come so far in a relatively short time.
– Julia Bandow
“Our greatest success is that we have come so far in a relatively short time,” Julia Bandow points out. “The plasma reactor might open up a new class of enzymes for commercial use.”
In the search for other enzymes that can produce valuable chemicals, the researchers are pursuing several strategies. Streptomyces, a group of soil-dwelling bacteria, also have hydrogen peroxide converting enzymes. However, the characterisation of the first three candidates hasn’t yet turned up any promising manufacturers of attractive products.
Another route to new enzymes could be through compost. Tests with model substrates, whose turnover is for example indicated by a colour change, revealed promising results. “We already know at what pH and temperature such reactions take place,” says Bandow. “But we haven’t yet identified which enzymes are responsible. That is the great unknown.”
adapted from Meike Drießen (RUB)
- Details
Plasmas as chemistry labs
Even though plasmas at atmospheric pressure are often only a few cubic millimetres in size, they pack quite a punch. This is because special non-equilibrium states can be set up in them, which facilitate physical and chemical processes that are not possible in any other environment. The plasma thus becomes a special kind of laboratory, where atoms and molecules can be excited without their surroundings heating up. “Such excitations could theoretically also be generated in a gas, but to do so we would have to heat it to several thousand degrees Kelvin. As a result, the molecules would decompose,” explains Professor Uwe Czarnetzki, Head of the Chair of Plasma and Atomic Physics at the Faculty of Physics and Astronomy. For many years, he and his team have been developing methods to explore the processes inside plasmas and to characterise the plasmas.
Plasmas boast a unique feature: electric fields can be used to supply energy to the electrons in the plasma; the electrons in turn interact with molecules such as nitrogen or carbon dioxide while transferring the energy to them. The molecules are excited, and this happens without the environment heating up in the process, as would be the case in a gas. The molecules that are excited to vibrate have a much higher reactivity than those in the ground state. Plasma can therefore change chemistry or even enable certain chemical processes in the first place. Consequently, plasma provides basic researchers with a unique opportunity to study the excitation of molecules and the associated chemistry beyond thermodynamic equilibrium. Uwe Czarnetzki is therefore primarily interested in the vibrational states of molecules in plasmas.
Analysing vibrations in molecules
The individual atoms of molecules – for example, the carbon and oxygen atoms in the carbon dioxide molecule – are not rigidly bonded to each other. The bonds between the atoms periodically deform in different ways. These oscillations can take place on several energy levels, i.e. with different frequencies that can be excited by light energy. This requires the frequency of the light to correspond to the difference in frequencies between two neighbouring energy levels. It is possible to deduce the number of absorbing molecules in the light beam from the decrease in the intensity of the light at this particular frequency. The Bochum-based physicists led by Uwe Czarnetzki and Dr. Dirk Luggenhölscher in Collaborative Research Centre 1316 “Transient Atmospheric Pressure Plasmas” are using this fact to analyse the vibrational states of molecules in plasmas, specifically in collaboration with Humboldt Fellow Dr. Yanjun Du. She wants to find out how many different states occur and how often.
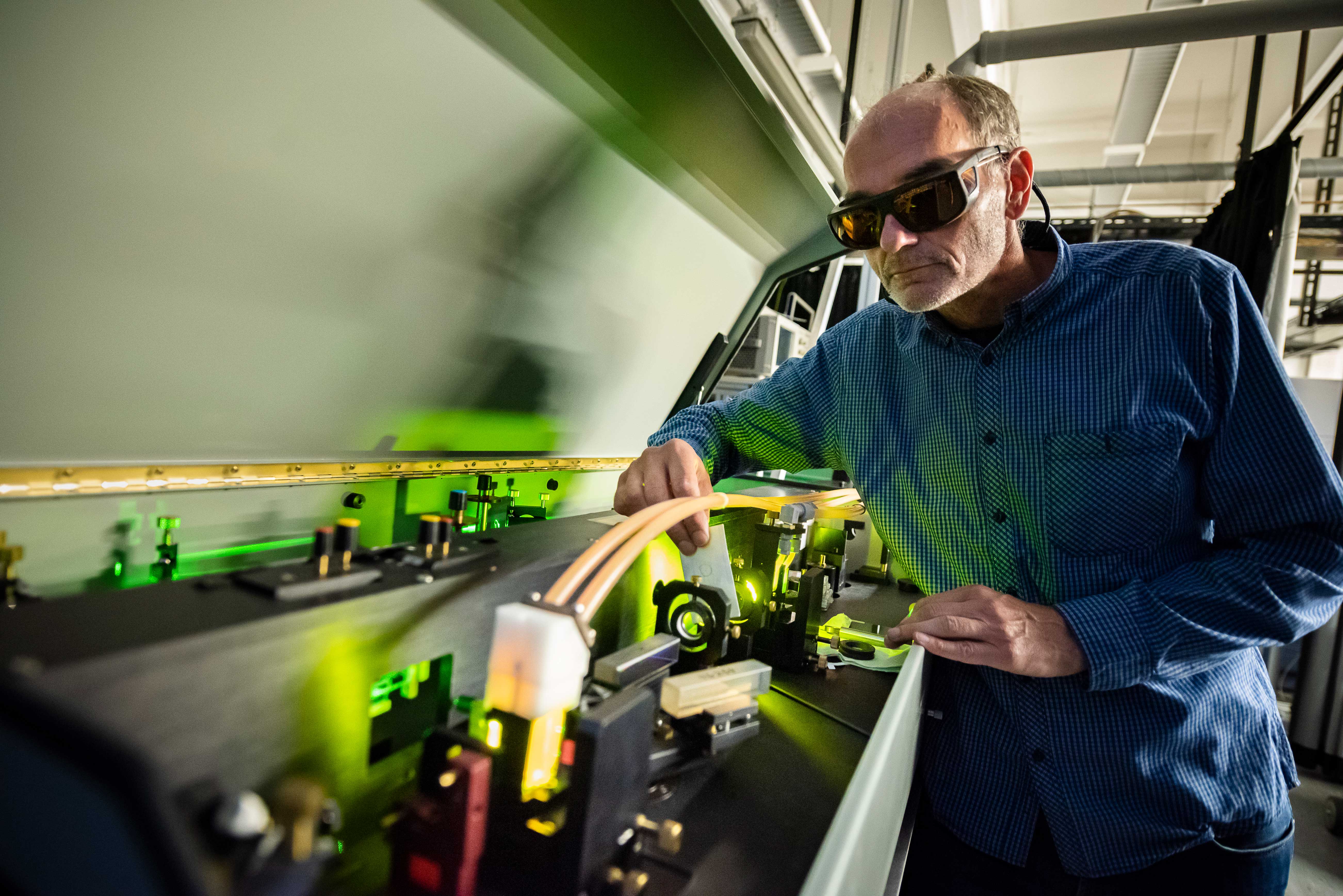
“Unfortunately, this method doesn’t work for all molecules or vibrations,” says Czarnetzki. That’s why he and his team, foremost among them Jan Kuhfeld, have refined the CARS method, short for Coherent anti-Stokes Raman Scattering. The complex laser method detects the otherwise forbidden transitions with high sensitivity, temporal and spatial resolution. In particular, the researchers revised the evaluation procedure for the theoretical calculation of the spectra so that they can also determine energy distributions that do not correspond to a thermodynamic equilibrium. “This is precisely the crucial point when using plasmas,” says Czarnetzki. The team also modified the laser system to detect all oscillation states simultaneously.
Understanding the mechanism behind excitation
The electric field is crucial to excite the oscillatory states. The crux of the matter is that a very high electric field is needed to generate the plasma, while a comparatively small field is needed for efficient vibration excitation.
Plasmas tend to do things that we don’t understand at first, but which are useful.
– Uwe Czarnetzki
At first, it was not clear why efficient oscillation excitation works despite this supposed contradiction. “Plasmas tend to do things that we don’t understand at first, but which are useful,” points out Czarnetzki. “Then, once we understand the mechanism, it turns out to be very mundane in retrospect.” Thanks to a new laser technique developed in Bochum by Dr. Nikita Lepikhin and his colleagues to measure the electric fields, the researchers finally understood what exactly happens when the plasma is created and the oscillations are excited.
First of all, one has to bear in mind how a plasma is created: To begin with, there is a gas to which energy is added in the form of electric current, until finally a high-energy state emerges in which a certain proportion of the gas is ionised. However, not all charges are generated at once. Initially, only some particles of the gas are ionised and accelerated, which in turn produces new charges. “In a few nanoseconds, an avalanche of charges forms and a high-density plasma is created,” outlines Uwe Czarnetzki. It is not possible to maintain such a high-energy state permanently, so the plasmas are operated in pulsed mode, i.e. switched on and off again and again, so to speak, typically a few thousand times per second.
Pulsed plasma solves supposed contradiction
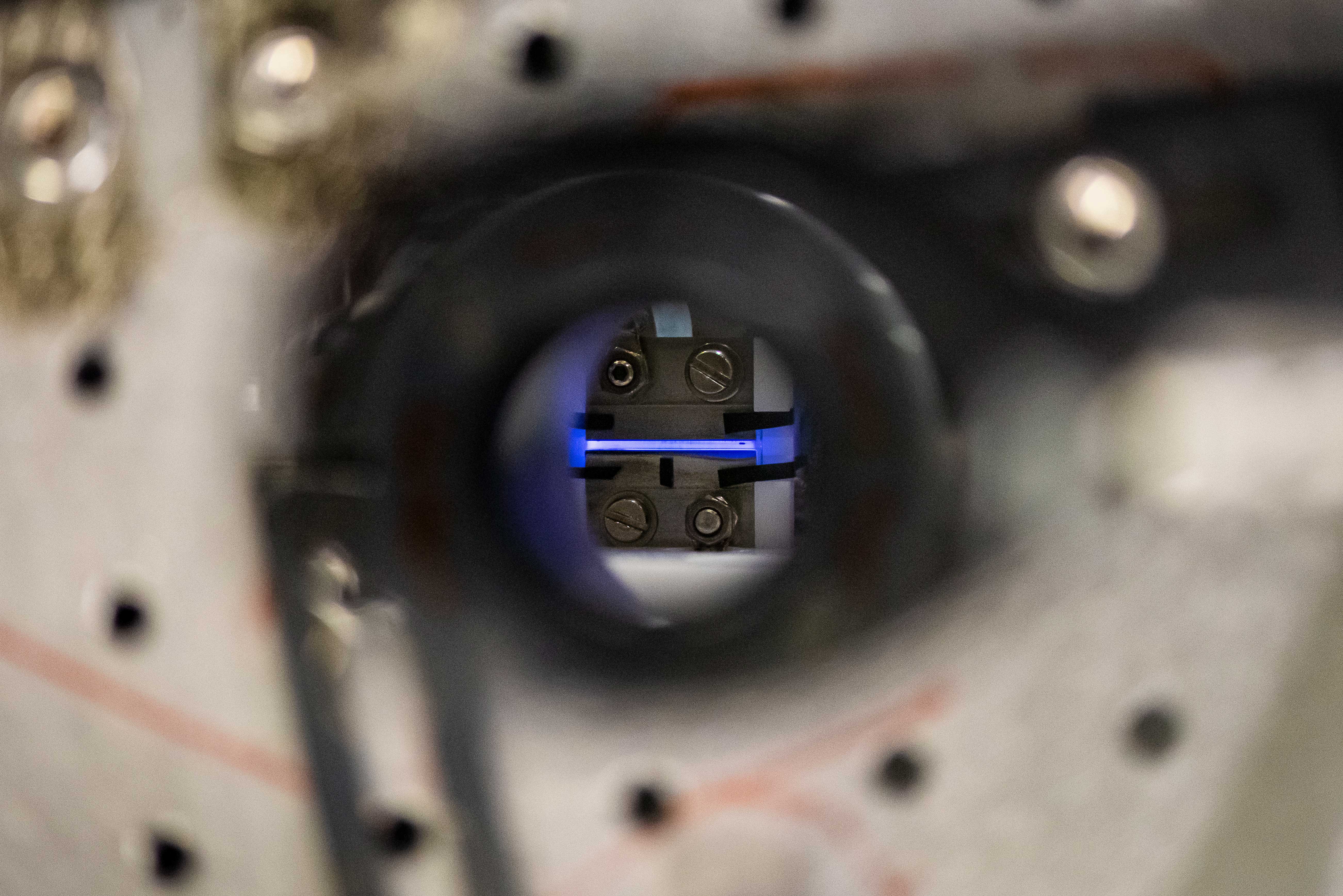
Due to the extremely fast pulsing of the discharge, the plasma solves the supposed problem of the different optimal electric field for plasma generation and oscillation excitation all by itself, so to speak. In the first nanoseconds after applying the voltage, there is a low plasma density, resulting in a high electrical resistance and thus a high field. Following strong ionisation, an exceptionally high plasma density is eventually achieved. This reduces the resistance and thus also the field. In the subsequent phase, the field is small and ideal for the excitation of oscillations. In addition, the charges discharged by the current flow are suitably replenished in a very thin transition region between the plasma and the electrode. By far the largest part of the high-density plasma, however, doesn’t generate any new charges, but only acts as a current conductor. Here, the electrons are gently accelerated in a weak field and give up almost all of the energy thus absorbed to the vibrational excitation of the molecules.
This already works well enough, at least in nitrogen molecules. In future, the physicists also intend to use this method to study CO2molecules. Initial measurements and theoretical considerations give them cause for optimism.
Combining different measuring methods
In the meantime, Czarnetzki’s team has also succeeded in combining the method for measuring the vibrational states with the method for measuring the electric fields in plasmas – a challenging task. “The smaller a plasma, the bigger the experimental setup required to study it,” points out Uwe Czarnetzki. “Our plasmas are so small that we don’t have room to insert a thick filament into them.”
It can be enough if a cable is laid incorrectly to get interference in the system.
– Uwe Czarnetzki
Laser technology solves this problem, but it requires complex experimental setups. Therefore, the experimental setups for measuring the electric fields and the vibrational states couldn’t simply be coupled. Instead, the physicists created two identical plasmas with great effort and attention to detail, on which they can run the measurements simultaneously and then pool the data. Each little detail made a difference. “It can be enough if a cable is laid incorrectly to get interference in the system,” says Uwe Czarnetzki. The fact that it was possible to obtain a coherent picture of the processes in the plasma for the first time by combining the two sensitive measurement methods is therefore one of the highlights of his research.
adapted from Julia Weiler (RUB)
- Details
The tailored wave
Boosting the efficiency of plasmas tenfold with the same energy input.
Plasmas are called the fourth state of matter: in the solid phase, their molecules occupy solid places, whereas there is some freedom of movement in the liquid phase and a lot of more freedom of movement in the gas phase. If more energy is supplied to a gas, the molecules break up and a plasma is created. The negatively charged electrons separate from the positively charged atomic nuclei and turn them into ions. These free electrons and ions can be accelerated by electromagnetic fields. If the fast electrons collide with other molecules, they can change them in turn by ionising or breaking them down. This process can result in different, sometimes short-lived reactive neutral particles and ions that may be useful for a variety of applications.
Smartphones, laptops, wafers
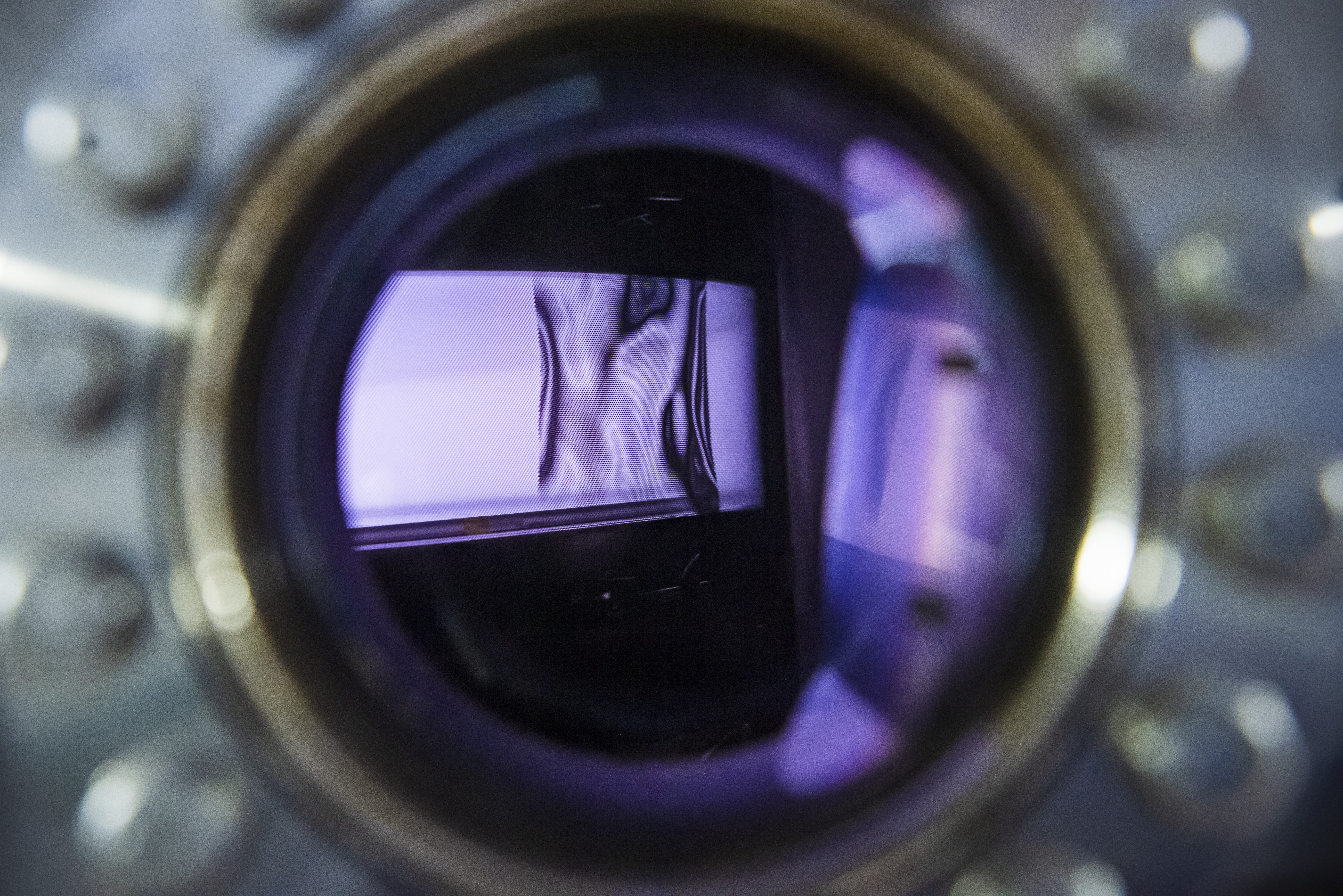
In the industry, plasmas are used, for example, to modify surfaces in a specific way, including coating spectacle lenses or displays or etching microscopic channels into silicon wafers. This results in fine structures in the nanometre range. “Every smartphone, every laptop contains components that have gone through such processes,” as Dr. Julian Schulze from the RUB Institute for Electrical Engineering and Plasma Technology outlines the use of plasmas in industrial applications. His aim is to better understand the details of processes in plasmas in order to render them more efficient. How is energy supplied to electrons and ions? How are they accelerated? How can this process be optimised?
In order to explore these questions, he concentrates on plasmas that are generated at room temperature, so-called low-temperature plasmas. They are often used for medical and industrial applications, because they do not damage or destroy their surrounding surfaces. In the medical field, this applies to e.g. human skin.
Such plasmas can, for example, be ignited between two electrodes, one of which is grounded, while a voltage is applied to the other. Applying the voltage leads to the generation of an electric field at the electrodes, which repels the negatively charged electrons from the surfaces while attracting positively charged ions. Because of the rapid movement of the electrons as a result of this acceleration, neutral particles can be excited by collisions with fast electrons. This ultimately makes plasmas glow. The acceleration of the ions to the surface can be used for surface modification.
Typically, a sinusoidal voltage is applied to the electrode – including industrial applications. This accelerates the electrons in a specific way. This, in turn, causes the plasma to emit light – which can be measured – at certain times and positions. Furthermore, positive ions reach the surfaces with a very specific energy distribution. However, Julian Schulze is not satisfied with this result: “Many electrons receive some of the energy that is fed in, but many of them do not receive enough to efficiently break up other molecules and, thus, produce high reactive particle densities. Also, the ion energy distribution at the interfaces cannot be controlled efficiently,” he points out. “We do not want to distribute the energy to electrons and ions with a watering can, but to deploy it in a more controlled manner so that fewer electrons receive more energy and the ion energy at interfaces can be precisely adjusted. This will enable plasmas to work more efficiently.”
The energy input to the electrons of the plasma can be controlled and improved
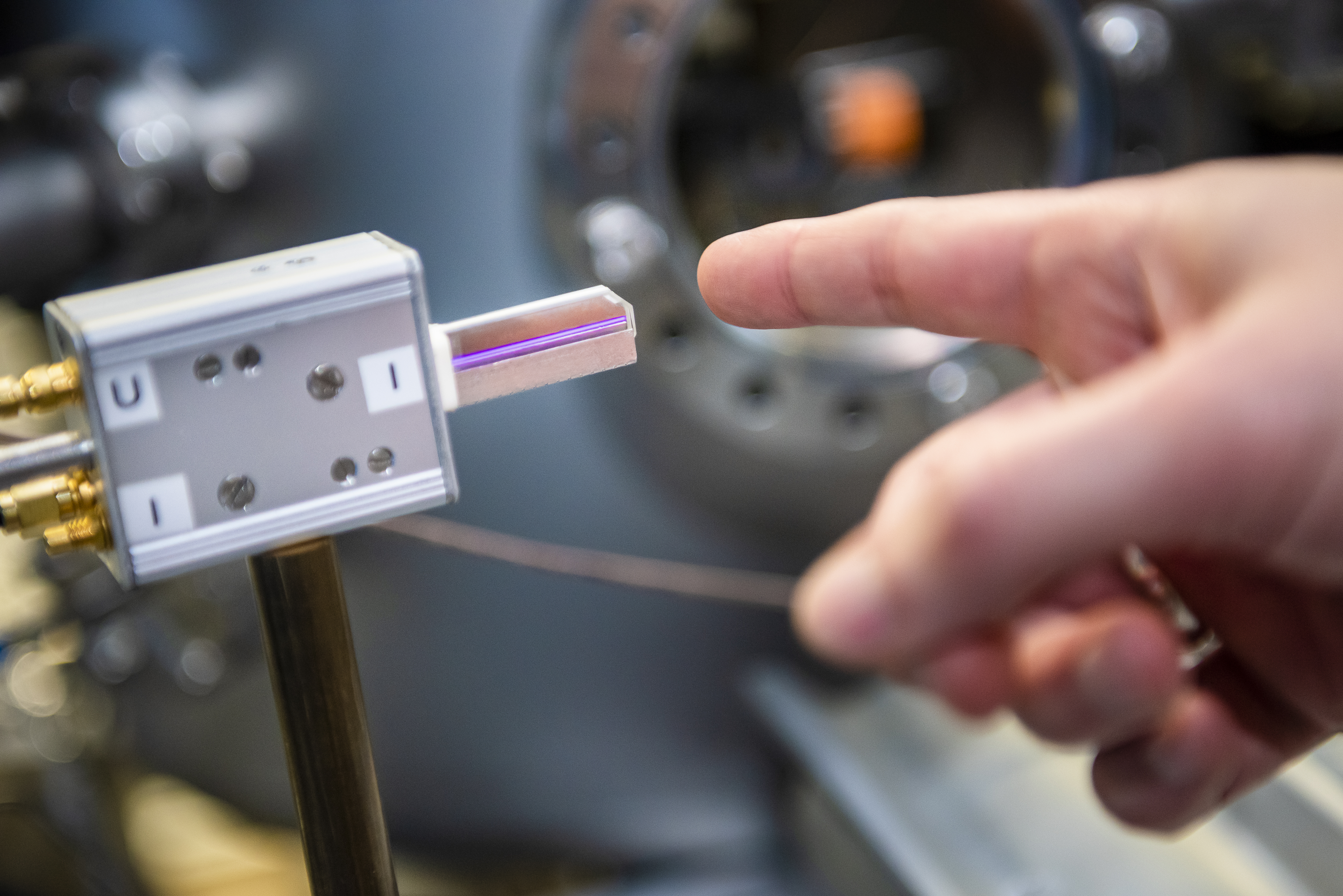
To this end, his team experimented with the form of the voltage waveform applied to the electrode and used in simulations. The process developed at RUB is called Voltage Waveform Tailoring, VWT for short. By superimposing several frequencies, different voltage waveforms can be generated, and the researchers have studied their effects on the plasma.
It emerged that, by tailoring this voltage waveform, the temporal and spatial distribution of the energy input to the electrons of the plasma can be controlled and improved. In the same way, the ion energy distribution at interfaces can be adjusted. “While in the case of the sinusoidal voltage many electrons are weakly accelerated at different points in time and over wide spatial areas, we have succeeded in using such optimised voltages to cause a much stronger acceleration of certain electrons at only one point in time within a period of the applied voltage and at specific spatial positions within the plasma,” explains Schulze. Thus, while the same amount of energy is dissipated, the amount of reactive species generated in the plasma increases. In principle, the situation is similar with ions: here, too, the acceleration of the charge carriers can be tailored with regard to time and space.
The mechanisms underlying these changes are the subject of in-depth studies. The effect of the voltage waveform on the plasma is rooted in the change in the relevant boundary zones near the electrodes, called plasma sheaths: the area in which a strong electric field exists, which is created by applying the voltage to the electrode, is a taboo zone for electrons; they are repelled. The positively charged, heavier, slower and colder ions, on the other hand, are attracted. The form of the applied voltage waveform modulates this boundary zone: it expands according to a pattern influenced by the voltage waveform and collapses again. “The electrons are kicked back into the plasma from the moving boundary region like a tennis ball from a racket,” as Julian Schulze illustrates one of several mechanisms that take place in the plasma. If the boundary layer is modulated so that it moves very fast, the electrons cannot keep up with it as quickly. “You have to imagine this in a similar way to when you pull a tablecloth away from under dishes so quickly that they simply remain where they were, even though you pull the floor away from beneath them. Then, you have to collect the dishes by hand, they do not follow the motion of the tablecloth,” says Schulze.
The positively charged ions perform the reverse motion, because they are accelerated towards the interfaces by the electric fields in the plasma boundary layer. Fast high-energy ions can be used to etch the interfaces, slower low-energy ions for coating. In any case, their energy and, thus, the processes on the surfaces can be tailored by VWT.
In order to find out exactly which mechanisms are at work when such tailored voltage waveforms are applied to the plasma, the engineers use e.g. high-resolution cameras to measure the electron dynamics. Moreover, laser measurements can be deployed to detect excited helium species and other reactive particles such as atomic oxygen, which are generated by collisions between neutral particles and energetic electrons. Since the excited helium particles absorb the laser radiation, it is possible to draw conclusions about the number of particles present by measuring the laser light that is transmitted by the plasma.
By superimposing two frequencies of the voltage applied to the electrode, the researchers measured a density of the particles that was more than five times greater. “Compared to applying the sinusoidal voltage, it is possible to increase the density of such particles tenfold and to control it by adjusting the voltage waveform,” Julian Schulze points out. Given the extent to which plasma is used in the semiconductor industry, the significance of this improvement is evident. “This is a billion-dollar industry,” says the researcher. “Every increase in efficiency has a huge economic impact.”
adapted from RUB, Meike Drießen, Donata Zuber
- Details
Plasma structures analysed in detail
Once the inhomogeneous character of plasmas became apparent, not everyone was pleased. However, this characteristic has some advantages, for example for the industry.
They are often invisible to the naked eye: the wafer-thin layers that are deposited on surfaces with the help of plasmas. For example, on architectural glass to control its reflectivity, on tools to protect them from wear and tear, or on plastics to make them more impermeable to gases. Plasma coatings have become indispensable in industrial applications. While surfaces can also be coated using chemical processes, this would sometimes require such high temperatures that the coated objects would melt. Plasmas, on the other hand, generate the required energy not through heat, but through the reactive particles they contain.
In a plasma, matter is partially or completely ionised. By applying electric fields to electrodes in a plasma chamber, the introduced gas, such as argon, can be ionised and the charged particles are accelerated towards a metal electrode. The ions impinging on the metal knock individual atoms out of the material, which are then deposited on a workpiece that is located across from it and is to be coated. The team of the Collaborative Research Centre SFB-TR 87 explores what exactly happens in the plasmas during such coating operations. The researchers have been studying the underlying processes for years.
Hype and valley of tears
“At our Collaborative Research Centre, we experienced both the hype surrounding high power pulsed plasmas for superior coatings a good ten years ago and the subsequent deep valley of tears,” recalls Professor Achim von Keudell, who holds the professorship for Experimental Physics of Reactive Plasmas at RUB. In 1999, the so-called High-power Impulse Magnetron Sputtering was established. The process uses fully ionised plasmas whose surfaces have a power density that pretty much equals that of rocket engines. In contrast to conventional plasmas, these high-power plasmas can’t be operated continuously, because they would destroy the materials of the plasma chamber. Therefore, they are repeatedly switched on and off, i.e. run in pulsed mode.
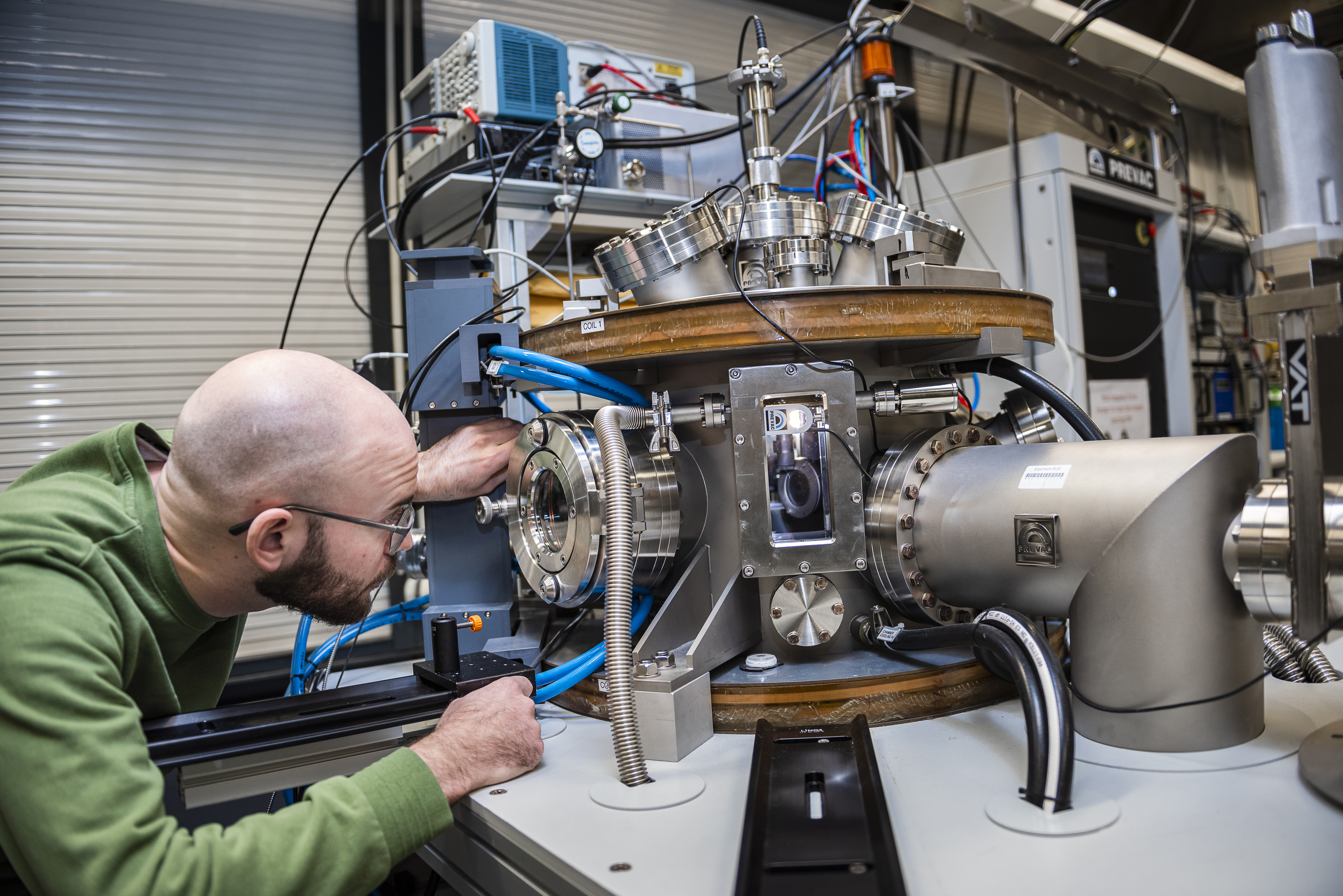
These dense plasmas can also be used to produce correspondingly dense high-quality coatings. Consequently, this technology immediately sparked interest in the industry. “Then, disillusionment hit,” says von Keudell. This is because the higher quality of the layers came at the expense of the coating rate, which in some cases was only 30 percent of those achieved by traditional processes at the same electrical power input.
While conventional processes mainly use uncharged atoms, radicals or molecules for coating, high-performance plasmas primarily produce ions. Since the particles are charged, they are heavily affected by external and internal electric fields. Due to the direction of these electric fields, a large proportion of the ions that travel towards the workpiece simply turn back halfway and fly back. This phenomenon is called the return effect and can’t be circumvented because it is simply due to the property of fully ionised plasmas. “For ten years, the plasma community has been struggling and failing to achieve both a high-quality layer and a high growth rate. It doesn’t work, you have to decide which is more important depending on the application,” elaborates von Keudell.
However, a larger number of the ionised particles make it to the other side through the electric fields and settle there as a layer than one would expect according to simple plasma models. This is thanks to another pheno
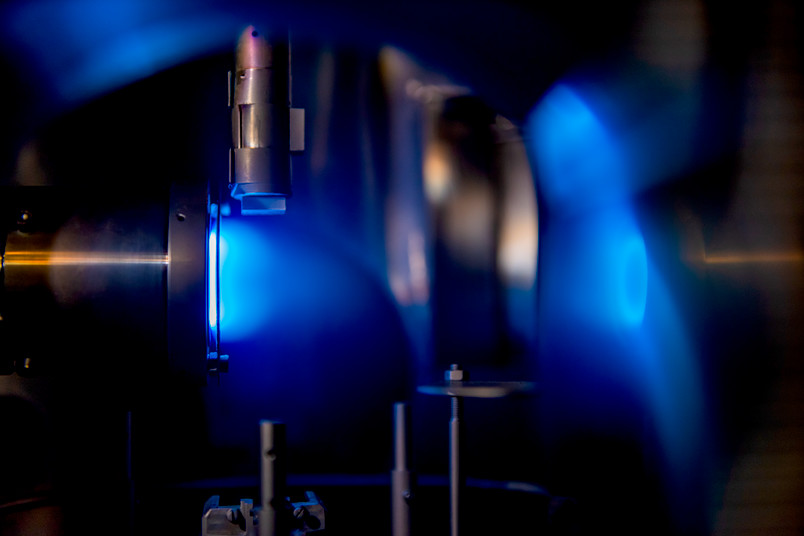
menon that the Bochum team has now researched in depth: although the high-power plasmas look very uniform when viewed with the naked eye, they actually contain structures that help the ions to get to the other side.
Croissants and comets
Viewed from above, the plasmas are toroidal, they resemble a glowing donut. This glow of the excited particles forms structures that move in circles at ten kilometres per second and can only be detected by high-speed cameras. “One of the first structures we observed in an aluminium plasma at that time looked like a comet flying backwards,” describes Achim von Keudell. In subsequent analyses, the Bochum-based researchers found that different structures were formed, depending on the type of plasma. In a titanium plasma, for example, not a single comet is formed, but rather something that looks like a croissant. The number of structures in the torus also changes depending on the plasma conditions. Because of the regularity and rotation of the structures, the term “rotating plasma spokes” has been adopted.
The description of these inhomogeneities in technical plasmas caused a stir in the research community, especially among scientists working in applied fields. Today, Achim von Keudell is convinced: “It was probably unfortunate that we basic researchers spoke of inhomogeneities or instabilities. A process engineer doesn’t like the sound of that.”
Stabile instabilities
It turned out that although these structures are created by instability at the beginning of each plasma pulse, the final structure is very stable. Structure formation is an essential property of high-power density plasmas and can’t be entirely prevented, but it doesn’t pose a problem for coating processes, either. On the contrary, it even helps to mitigate the return effect. Without the inhomogeneities, there would be no efficient layer growth at all in fully ionised plasmas, because many ions deflected by the electric fields would never reach the surface.
Because the luminous plasma spokes are electrically charged, they don’t interlock; once the structure is formed, it is stable. And because it moves at high speed, it does not affect the coating finish. Only under certain conditions can the structures freeze in their motion, which would indeed lead to an uneven coating. “In the meantime, however, we have understood the plasmas to such an extent that we know how to choose the parameters to prevent such an outcome,” says Julian Held, PhD researcher at the Chair of Experimental Physics II. “We can even specifically grow certain structures in the lab.”
Julian Held has perfected the method. He developed a technique that allows him to analyse structures in a plasma synchronised with high-speed cameras. Thus, he can also render the different plasma components visible separately from each other, such as the glow of certain atoms or ions, and correlate their movements in time and space. “For years, we had tons of individual images but never knew how to superimpose them,” Held recalls. “This project was an essential step in creating detailed plasma models.”
- Details
The universe is more homogeneous than expected
Recent results of the Kilo-Degree Survey have shown that matter in the universe is about ten percent more evenly distributed than predicted by the standard model of cosmology. An international team, led by astronomers from the Netherlands, Scotland, Great Britain, and Germany - with the participation of the Ruhr-Universität Bochum - describes the results in five articles, three of which were published on a preprint server on 31 July 2020. They are also submitted for publication in the journal Astronomy and Astrophysics.
The data were recorded with the European Space Organisation's Very Large Telescope Survey Telescope (VST) on Cerro Paranal in northern Chile. The resulting map covers five percent of the extragalactic sky and includes 31 million galaxies, all of which were included in the analysis. They are up to ten billion light years away, and their light was emitted when the universe was only about a quarter of the age it is today.
Using the galaxies, the research consortium produced a map of the distribution of matter in the universe. To do this, the scientists used the so-called weak gravitational lensing effect: light from distant galaxies is deflected and distorted on its way to Earth by the gravitational effect of large accumulations of matter such as galaxy clusters. Based on this effect, the clumping tendency of matter can be determined - namely visible matter, gases and invisible dark matter, which makes up about 85 percent of the total matter in the universe.
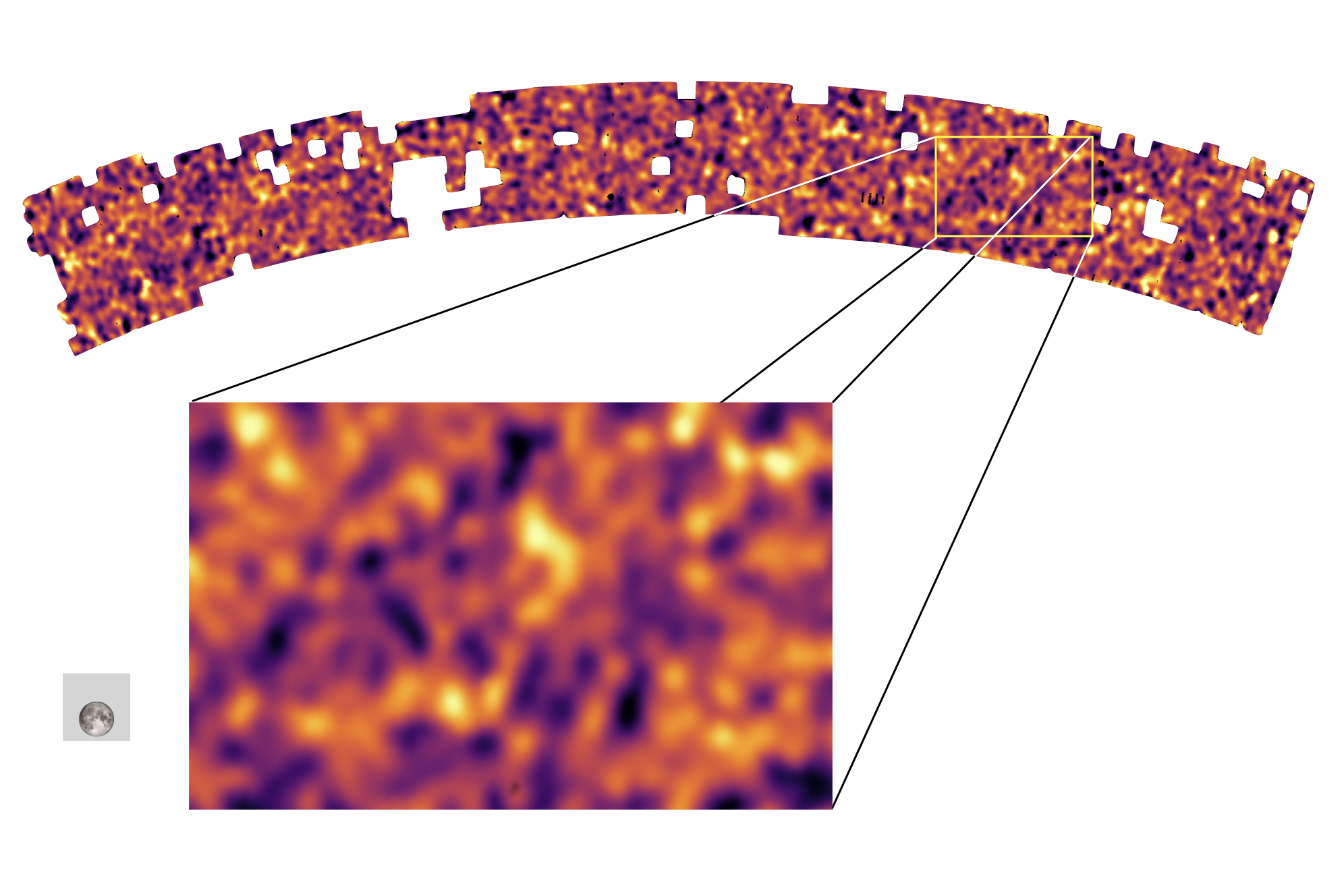
Over time, the gravitation of matter causes the universe to become less and less homogeneous. Areas with slightly more mass than average attract matter from their surroundings. Thus the differences in distribution become greater and greater. At the same time, the expansion of the universe counteracts this effect. Both processes are driven by gravity and are therefore suitable for putting the standard model of cosmology to the test. The equations predict precisely how much the density of matter will change over time.
However, the data from the Kilo-Degree Survey reveal a discrepancy: the universe is ten percent more homogeneous than it would appear to be according to the Standard Model. "The Standard Model of Cosmology has described all the cosmological observations we have made for the past 20 years. However, it is somewhat unsatisfactory that we have to accept mysterious substances such as dark matter and dark energy. That's why we're trying to test this model as best we can," says Prof. Dr. Hendrik Hildebrandt, head of the Observational Cosmology group at the Ruhr University Bochum.
The current analysis could indicate that the Standard Model is cracking. It is not the first discrepancy, even the so-called Hubble constant, which represents the expansion rate of the universe, does not match the model's predictions. "These discrepancies could of course be caused by systematic measurement errors," admits Prof. Dr. Catherine Heymans (University of Edinburgh), who together with Hendrik Hildebrandt heads the German Centre for Cosmological Lensing at the RUB, where she also holds a guest professorship. "But the measurements are becoming more and more precise, so that this is becoming increasingly unlikely," Heymans continues.
The researchers are not yet able to assess whether the Standard Model will eventually have to be replaced by a completely new theory, for example by replacing Einstein's General Theory of Relativity. "There are many theories that try to explain the measurements with new physics," says Hendrik Hildebrandt. "As an observing cosmologist, you try to remain impartial and make the measurements as accurate as possible without theoretical prejudice. One thing is clear, we live in exciting times!"
In one or two years the final map of the Kilo-Degree Survey will be available, with all the observations made in the project. It will be another 30 percent larger than the current map.
Meanwhile, two other projects, one in the US and one in Japan, are working on similar analyses based on observational data. From 2022, even better measuring technology will be available: the Ruby telescope, which is 60 times more powerful than the VST, and the Euclid satellite, which will take much sharper images outside the atmosphere.
The Kilo-Degree Survey is an international project led by astronomers in the Netherlands, Scotland, England and Germany. The project coordinator is Prof. Dr. Koen Kuijken from the Leiden Observatory in the Netherlands. Other partners come from Italy, Australia, Poland, the USA, and China.
adapted from Julia Weiler, RUB